Staffan Lindgren | August 22, 2020
.
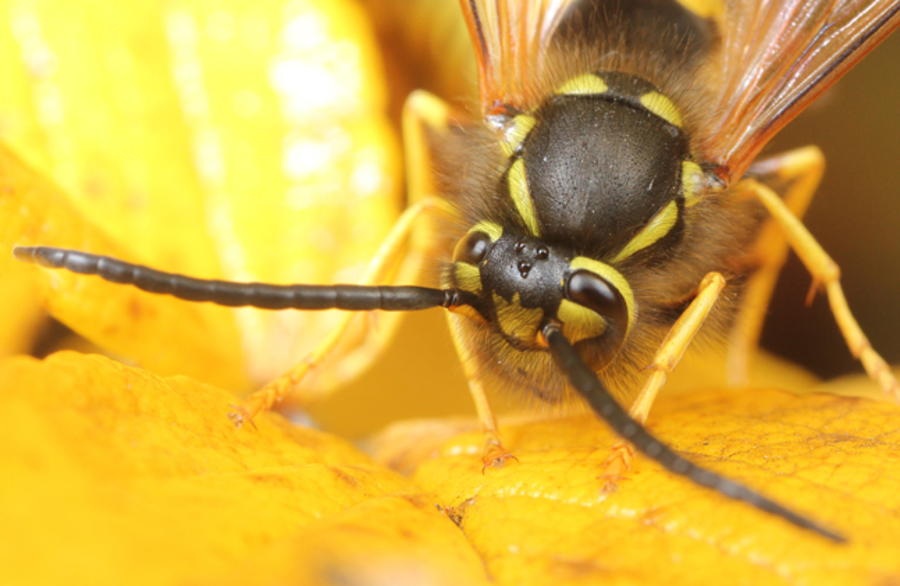
Vision is something that most of us value immensely. Humans are largely visual beings. Our eye sight is a primary sense organ by which we experience the world. Our other senses (smell, taste, touch and hearing) tend to be less refined, although much of that is because of lack of use. For example, some visually impaired or blind individuals can describe objects by sound in a way similar to what toothed whales and bats do https://www.sciencedaily.com/releases/2017/06/170626093624.htm, showing that there is enormous potential for expanding at least some of our secondary senses. Photoreception of one type or another has likely been present for at least 1 billion years, although the first true, fully formed eye (a compound eye) known from the fossil record dates back to the early or middle Cambrian (Schwab 2018). However, protists have been around for well over one billion years, and some have surprisingly advanced eyes, indicating that the evolution of eyes started well before the Cambrian.
Extraocular photoreception. Even animals with well-developed eyes frequently have various types of extraocular photoreception (Cronin and Johnsen 2016). Photosensitive receptors evolved early, and some types can be found in some very “primitive” animals. Unicellular protists emerged 1.7 – 2 billion years ago (Schwab 2018), and light sensitive structures may have appeared within a few hundred million years of that. The earliest examples are found among prokaryotes (bacteria and cyanobacteria [aka blue-green algae] which lack a cell nucleus) and various eukaryotes (with a cell nucleus), i.e., in some of the 45 or so Phyla of single-celled protists. Some ciliates appear to have both somatic and ciliary photosensitive proteins, enabling them to perceive and respond to light (Nakaoka et al. 1991). In the so-called phytoflagellates, i.e., protists which photosynthesize such as Chlamydomonas and Euglena, the photosensitive protein is concentrated in what may be considered the first step in the evolution toward true eyes. Eye spots have light sensitive proteins positioned above a pigment layer but cannot determine the direction of the light source directly, but by sensing sequential changes in light intensity they can move toward or away from light (phototaxis). There are 10 different types of eyes, the majority of which are found among invertebrates (Warrant 2017a), but the evolution and functions from the simple eye spot to the vertebrate eye are still being unraveled by scientists.
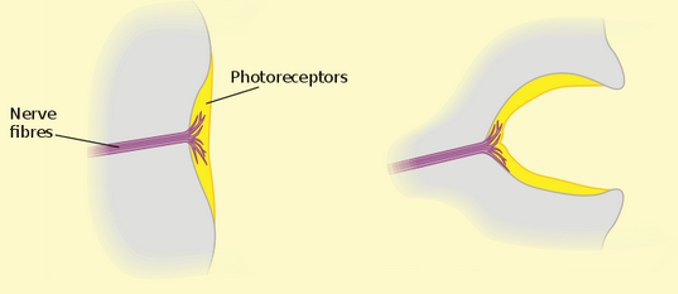
Pit eyes. Flatworms (Phylum Platyhelminthes) include parasitic tapeworms and flukes, but the group of interest here is the Turbellaria, e.g., the well-known freshwater Planarians in the genus Dugesia. These fascinating creatures are commonly used in high school and university biology labs to demonstrate regeneration of body parts, but it is their eyes that we are interested in here. The anatomy of the pit eyes of a Planarian is essentially a cup with light sensitive receptors at the bottom (Warrant 2017a). This allows the animal to detect light levels, but not to form images. They can determine direction of light source since each receptor in the pit receives light from different directions, giving them a crude spatial vision, so pit eyes are considered true eyes. Interestingly, just like the prokaryotes, some species can respond to light even if their head with the eye cups is removed. This indicates that in addition to the pit eyes, these Planarians have some kind of secondary photo receptor (Shettigar et al. 2017). Pit eyes are also found in sea jellies and sea stars. Interestingly, recent research has shown that sea stars actually can form crude images (Garm and Nilsson 2014, Birk et al. 2018).
The next step in the evolution of eyes is the pinhole eye. It is now quite rare, found only in a few molluscs, notably the six species of Chambered Nautilus (Nautilus and Allonautilus) and abalones in the genus Haliotis. The pinhole eye is a cavity lined with a retina that receives light from a pinhole opening (Warrant 2017a). Pinhole cameras operated on the same principle, and ophthalmologists still use pinhole tests to assess visual acuity. A pinhole eye produces a fairly sharp image depending on the size of the pinhole. You can see this effect for yourself. For example, I have corrective lenses, so without glasses what I type here will be blurry. If I make a pin prick using a thumb tack in a piece of paper and look at the screen through that, I get a sharp image, albeit of a very small part of the screen!
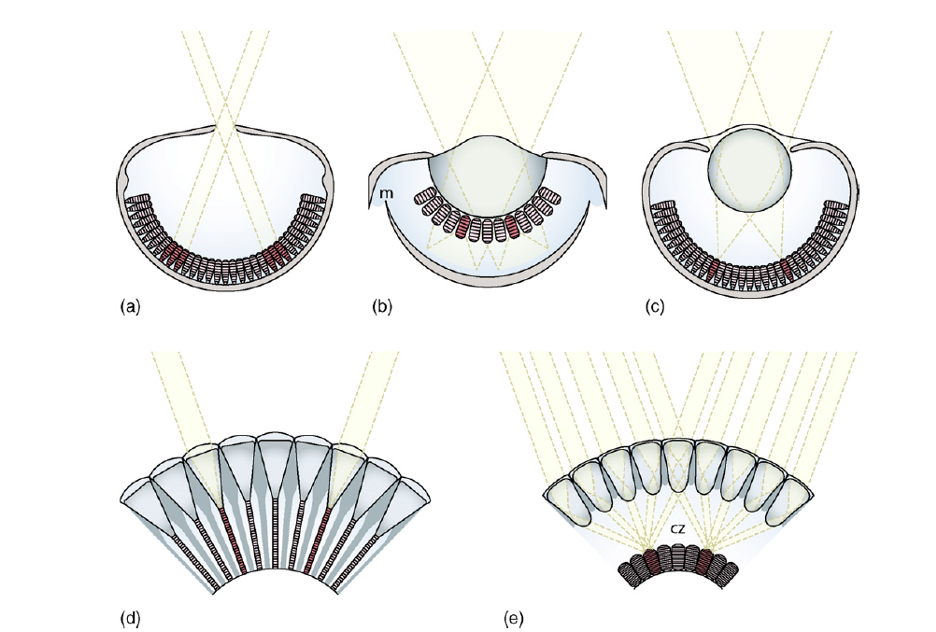
The next type of eye is also prevalent among molluscs, notably scallops and clams, as well as some ostracods (Crustacea). This is the complex concave mirror eye, in which weakly defracted light by a gelatinous lens passes through a distal retina and is reflected by an underlying mirror (tapetum) focusing the light on the proximal retina (Warrant 2017a). Since some poorly focused light has already been absorbed the resulting image has poor contrast, but scallops can detect the movement of fairly small objects. The puzzling presence of two retinas was explained by Palmer et al. (2017) who found that the distal retina focuses light coming from the front, but as the angle increased, the focal plane moved toward the proximal retina. Finally, the optic nerves of nearly all eyes on a scallop project to the same brain center, visual information from each eye may be integrated across the whole visual field (Palmer et al. 2017, Warrant 2017a).
Compound eyes may be the most widely distributed type of eye in the animal kingdom. Compound eyes appeared about 500 million years ago and have been conserved ever since (Schoenemann et al. 2017). Two separate evolutionary types are known, one derived from a common ancestor with a crystalline cone lens (e.g., insects and crustaceans), and a second derived from one with a corneal lens (e.g., millipedes and centipedes, and horseshoe crabs) (Nilsson and Kelber 2007). The former is capable of colour and polarization vision. Compound eyes consist of a number of individual ommatidia, each with its own lens. There are two types of compound eyes, the apposition eye and the superposition eye. In apposition eyes each ommatidium is isolated by a pigment sleeve so it receives light only from its own lens. These are present mostly in diurnal animals. In the superposition eye the pigment sleeve can be withdrawn to allow light from several ommatidial lenses to reach the light sensitive rhabdom (Warrant 2017b). The collection of light can be further enhanced by the presence of a reflective tapetum, which is why the eyes of nocturnal moths glow when light is shone on them. The number of ommatidia varies with species from 5 in an arctic isopod to 30,000 in some dragonflies. The higher the number of ommatidia, the higher the resolution of the resulting image.
Colour vision varies considerably among different taxa (Briscoe and Chittka 2001). Most insects have spectral sensitivity maxima in UV, Blue and Green. Butterflies have an additional spectral peak in red, whereas many species in several other Orders have only monochromatic sensitivity. Stomatopods (mantis shrimps) have a different type of colour vision than other animals. Their compound eyes are also unique, comprising two hemispheres separated by a midband. The stomapod eye is organized so that each eye sees only a narrow strip, so the stalked eyes are in constant motion (Marshall et al. 2007). In addition, each eye functions independently of the other. Remarkably stomapods have monocular stereo vision (they can judge distance with one eye), polarized light vision, and some species have as many as 12 different photoreceptors with different spectral sensitivity. Yet their ability to discriminate among spectra separated by 12-25 nanometers is relatively poor (Thoen et al. 2014). Whereas colour vision in most animals depend on spectral discrimination, stomapods essentially recognize colours by scanning objects.
Camera eyes are eyes where a lens, sometimes overlaid with a cornea, focuses the light on the retina. Virtually all vertebrates (except some cave or subterranean species that are secondarily blind) have camera eyes, including humans (Warrant 2018). In addition, camera eyes are found in some molluscs, cnidarians, arthropods and annelids (Warrant 2018). Even a dinoflagellate, a unicellular protist, possesses a so called ocelloid consisting of a pigment cup with a retina-like structure and a lens-like structure (Schwab 2018). In invertebrates the different components are derived from different tissues than in vertebrates, suggesting convergent evolution (i.e., the formation of similar function and structure from different origins). The eyes of cephalopods (squid, octopus and cuttlefish) are remarkably similar to vertebrate eyes. However, they have only one visual pigment, and have been assumed to be colour blind (Hanke and Kelber 2019). This is interesting, as octopus are extremely good at matching their skin to the surrounding environment. The octopus eye is able to focus by moving their almost spherical lens in the same way a camera lens focuses, whereas we focus by deforming the lens. Also, while we have a blind spot because the optical nerve passes through the retina, octopus do not as their optical nerves go around the retina. In addition, the octopus eye has more photoreceptors than human eyes. There are also some information processing differences.
Perhaps the most fascinating camera-type eye is found in box jellies (Phylum Cnidaria, Class Cubozoa). Box jellies are better known for their incredibly potent venom, which kills more people than sharks, e.g., an estimated 20-50 fatalities a year happens in the Philippines alone (Lippman et al. 2011)! Box jelly medusae (bells) differ from those of regular sea jellies (Class Scyphozoa) in having tentacles clustered in four corners, giving a somewhat box-like appearance. Box jellies are active hunters, and they are efficient swimmers, some traveling at up to 6 km/hour. Near the lower edge on each side are pendant structures called rhopalia, which houses balancing and visual organs. Each rhopalium contains six eyes, for a whopping total of 24. These are of four morphologically different types, two of which are camera-type, and two pit eyes (O’Connor et al. 2009). Remarkably, the camera eyes of box jellies appear closer to vertebrate than to invertebrate eyes (O’Connor et al. 2009). The lens has been shown to be capable of focusing light on the retina remarkably well, although visual acuity is not as good as in vertebrates. Nevertheless, it should be noted that Cnidarians are very simple organisms, lacking a brain, and having only two germ layers (diploblasts) as opposed to three of “higher” organisms (triploblasts). Yet Cubozoans possess eyes which for all intents and purposes are similar to our own.
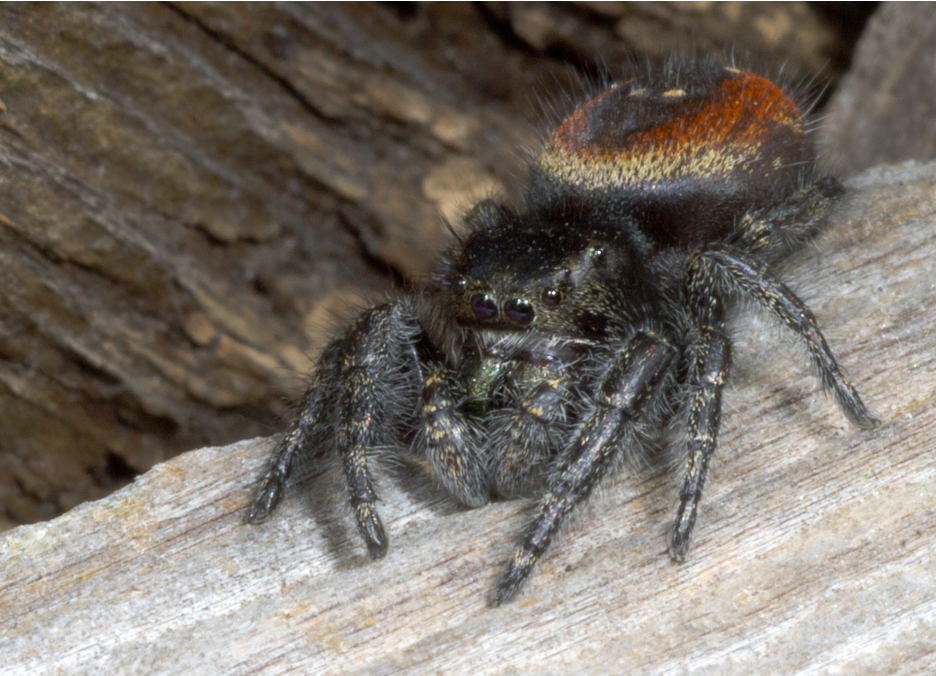
The above description is an attempt to simply give a taste of the wide variety of vision that occurs in nature. I have left out many details and examples, all of which are remarkable. I have tried to provide key references, but again many key studies have no doubt been missed. I am not an expert in vision so several misrepresentations are likely in the text above. Nevertheless, I hope this blog gives a sense of the incredible power of evolution through natural selection.
.
References
Birk, MH, ME Blicher and A Garm. 2018. Deep-sea starfish from the Arctic have well-developed eyes in the dark. Proceedings of the Royal Society B: Biological Sciences 285: 20172743 DOI: 10.1098/rspb.2017.2743
Briscoe, AD and L Chittka. 2001. The evolution of color vision in insects. Annual Review of Entomology. 46: 471–510
Cronin, TW and S Johnsen. 2016. Extraocular, Non-Visual, and Simple Photoreceptors: An Introduction to the Symposium. Integrative and Comparative Biology. 56: 758–763, DOI: 10.1093/icb/icw106
Garm, A, and D-E Nilsson. 2014. Visual navigation in starfish: First evidence for the use of vision and eyes in starfish. Proceedings of the Royal Society B: Biological Sciences 281: 20133011 DOI: 10.1098/rspb.2013.3011.
Hanke, FD, and A Kelber. 2019. The Eye of the Common Octopus (Octopus vulgaris). Frontiers in Physiology, 10: 1637, DOI: 10.3389/fphys.2019.01637
Lippmann, JM, PJ Fenner, K Winkel, and L-A Gershwin. 2011. Fatal and Severe Box Jellyfish Stings, Including Irukandji Stings,in Malaysia, 2000–2010. Journal of Travel Medicine 8: 275–281. DOI: 10.1111/j.1708-8305.2011.00531.x
Marshall, J, TW Cronin and S Kleinloge. 2007. Stomatopod eye structure and function: A review. Arthropod Structure & Development 36: 420-448, DOI: 10.1016/j.asd.2007.01.006
Nakaoka G, R Tokioka, T Shinozawa, J Fujita, and J Usukura. 1991. Photoreception of Paramecium cilia: localization of photosensitivity and binding with anti-frog-rhodopsin Ig. Journal of Cell Science 99: 67-72.
Nilsson, D-E, A Kelber. 2007. A functional analysis of compound eye evolution. Arthropod Structure & Development 36: 373-385, DOI: 10.1016/j.asd.2007.07.003
O’Connor, M, A Garm and D-E Nilsson. 2009. Structure and optics of the eye of the box jellyfish Chiropsella bronzie. Journal of Comparative Physiology 195: 557-569, DOI: 10.1007/s00359-009-0431-x
Palmer, BA, GJ Taylor, V Brumfeld, D Gur, M Shemesh, N Elad, A Osherov, D Oron, S Weiner, and L Addadi 2017. The image-forming mirror in the eye of the scallop. Science, 358: 1172-1175 DOI: 10.1126/science.aam9506
Schoenemann, B, H Pärnaste, and ENK Clarkson. 2017. Structure and function of a compound eye, more than half a billion years old. PNAS 114: 13489-13494, DOI: 10.1073/pnas.1716824114
Schwab, I. 2018. The evolution of eyes: major steps. The Keeler lecture 2017: centenary of Keeler Ltd. Eye 32, 302–313, DOI: 10.1038/eye.2017.226
Shettigar,N, A Joshi, R Dalmeida, R Gopalkrishna, A Chakravarthy, S Patnaik, M Mathew, D Palakodeti1, and A Gulyani. 2017. Hierarchies in light sensing and dynamic interactions between ocular and extraocular sensory networks in a flatworm. Science Advances 3: e1603025, DOI: 10.1126/sciadv.1603025
Speiser, DI, ER Loew, and S Johnsen. 2011. Spectral sensitivity of the concave mirror eyes of scallops: potential influences of habitat, self-screening and longitudinal chromatic aberration
Journal of Experimental Biology 214: 422-431; DOI: 10.1242/jeb.048108
Thoen, HH, MJ How, T-H Chiou and J Marshall. 2014. A different form of color vision in mantis shrimp. Science 343: 411-413 DOI: 10.1126/science.1245824
Warrant, E. 2017a. Invertebrate Vision, In Roitberg, BD (Editor), Reference Module in Life Sciences, Elsevier, 180 pp. DOI: 10.1016/B978-0-12-809633-8.01303-0
Warrant, EJ. 2017b. The remarkable visual capacities of nocturnal insects: Vision at the limits with small eyes and tiny brains. Philosophical Transactions of The Royal Society B Biological Sciences 372: 20160063, DOI: 10.1098/rstb.2016.0063
Warrant, EJ. 2018. Visual optics: Remarkable image-forming mirrors in scallop eyes. Current Biology. 28: R262-R264 DOI: 10.1016/j.cub.2018.01.079